Study of Massive Floating Solar Panels over Lake Nasser
Recently, the technology of floating photovoltaic panels has demonstrated several advantages over land installations, including faster deployment, less maintenance cost, and higher efficiency. Lake Nasser is the second largest man-made freshwater lake in the world with a surface area of almost 5000 square km. Being in one of the hottest areas in the world, evaporation of water causes loss of very precious and scarce resources: freshwater. Fortunately, the lake is also located in a very rich area in solar energy. This paper presents a study to utilize Lake Nasser’s surface for massive production of solar energy, while significantly reducing the loss of water by evaporation from the lake surface. The project has the potential to be one of the largest producers of low-cost clean electric energy in the world for Europe and the Middle East and North Africa (MENA) region, especially with the ongoing efforts to connect the North African countries with the European super power grid. The study shows that the first phase of the project is expected to deliver about 16% of European need of electricity and save about 3 billion m 3 of freshwater. The subsequent phases will provide low-cost green energy to replace the combustible fuels in Europe by 2045, while saving up to 10-12 billion m 3 of freshwater lost by evaporation from Lake Nasser.
Introduction
The European Union (EU) has established environmental protection and migration to renewable energy as strategic goals [1]. The European revised renewable energy directive 2018/2001/EU has set a target for the renewable energy (hydropower, solar, and wind) share to meet or exceed 32% by 2030 [2].
In 2016, Europe consumed almost 3100 TWh/y [3]. The combustible sources accounted for 48.7% of the generated electricity. The wind energy and solar energy accounted for 9.7% and 3.5%, respectively. However, the recent decline of the land wind power generation in Germany sparks concerns about the potential of wind power in meeting the European environmental goals [4].
A study conducted by Fraunhofer Institute for Solar Energy Systems (ISE) examined the technical potential of deploying solar technologies in North Africa and its ability to meet the European need for electricity [5]. The report estimated electricity demand of North Africa and Europe to be 5850 TWh in 2050 and proposed to establish a super electric power grid to integrate the solar energy production in North Africa with Europe.
Egypt has already embarked upon a number of large-scale solar electricity projects. For example, the Benban project, the 4th largest solar power plant in the world, is aimed at establishing a solar park over 37.2 square kilometers, in the southern part of Egypt. The Benban project has the potential to produce almost 1.7 GWp, with a total annual production capacity of 3.8 TWh [6].
Recently, the floating photovoltaic (FPV) panels have been demonstrated in several areas worldwide [7, 8]. The FPV technology promises to provide solar energy cheaper than PV land installation because it has higher efficiency than land installations due to the cooling effect of the water mass. FPV installations are also faster to deploy and require less cleaning compared with the cleaning/maintenance cost for land installations in dry dusty desert areas [9].
Lake Nasser in Egypt has a surface area of about 5000 km 2. It is located in one of the richest areas in solar energy in the world. In this paper, we present a study for a massive deployment of floating PVs over Lake Nasser to provide low-cost green electricity for Europe and North Africa, integrated with the European-North Africa super grid [5]. The coverage of the lake surface could also save as high as 12-16 billion m 3 /year of evaporated water, enough to alleviate some of the impacts of the Ethiopian renaissance dam on Egypt. The presented study is aimed at providing a comprehensive account of the key factors to help all the interested parties to develop the best economical and engineering strategies of such a terascale project, and it is to be the starting point for further economic, engineering, social, and environmental studies. The next section provides a review on Lake Nasser’s geography, water hydrology, and climatology factors. The following sections cover water evaporation problem and water saving, solar energy potential, and the estimated cost based on the current commercial technology of PV and the technology trends. Next, we discuss various options for energy storage technologies and mitigation of possible impacts of the project on fishery and ecology.
Lake Nasser
Lake Nasser resulted from the Egyptian High Dam construction, during the period from 1958 to 1971 [10]. The lake is the High Dam’s reservoir, extending roughly from 21 ° 12
32 N and 30 ° 40 32 E in Sudan to 23 ° 58 25 N and 32 ° 51 43 E in Egypt [11]. The Egyptian part is known as Lake Nasser (see Figure 1), while the part in Sudan is known as Lake Nuba. However, 83% of the lake water exists on the Egyptian side. The long reservoir has 100 side major arms or bays called Khors; their number on the eastern shore is higher than that on the western shore. The reservoir is surrounded by rocky desert terrain. To the west is the great Sahara Desert, while the Eastern Desert side stretches to the Red Sea, with several shore side plateaus of 50-100 meters above the water level. The average width of the lake is 12 km, the average depth is 25 meters, and it is as much as 107 meters (350 feet) deep at the dam’s face [11].
2.1. Hydrology and Water Management
The knowledge of the seasonal and yearly variations in the surface level of Lake Nasser is essential for proper design of the mooring systems of FPVs. The total capacity of the reservoir is reported to be about 162 cubic km [11], consisting of a dead storage of 31.6 cubic km (85-147 m water level above sea level (ASL)), the active storage of 90.7 cubic km (147-174 ASL), and the emergency storage for flood protection which is 41 cubic km (175-182 m ASL); see Figure 2. The water year starts on August 1st, at which the water level is kept at 175 m ASL. When the water level upstream reaches an elevation between 178 m and 183 m, excess water is directed to a dipped landform known as Toshka depression and if necessary to emergency spillways. The maximum retention at 180 m ASL is obtained in November. The water levels then continue to decrease from January to July as water is discharged for normal nation’s consumption.
According to data from the Ministry of Water Resources, the relationship between both the water surface area and the perimeter of the lake versus the water level is depicted in Figure 3.
of the lake is related to the water level by the empirical formula
is the average monthly water level [13].
Several studies were conducted using satellite remote sensing data to assess the changes in the water level [14, 15] and water volume [16–18]. These studies helped as well in estimating the annual water loss by evaporation from the lake. The mean yearly variation in the water surface level based on records from 1992 to 2018 is about 6 meters [15], as shown in Figure 4.
2.2. Climate
The average minimum and maximum temperatures at Aswan city (about 7 km north of the High Dam) are shown in Figure 5. The figure shows that the average maximum temperature in summer is 42°C degrees while the average minimum temperature in winter is about 10 degrees [19]. Clearly, the selection of the construction material and the design of the electronic components for the FPVs need to consider the full range of the environmental temperature in the Lake Nasser region.
A study of climate records of the Aswan region from 1955 to 2013 reveals no significant changes for the climate due to the construction of the High Dam. It also concluded that the temperature at 2 m high is the main contributing factor to evaporation losses [20].
The wind speed has a mild monthly average of about 3.8 meters/s, as shown in Figure 5, which is about 13.7 km/h [20]. A summary of seasonal statistics of wind speed is shown in Table 1. The wind direction is mainly north as shown in Figure 6.
The wind speed and direction are important factors in the design of tilted PVs and the design of the power and communication towers and for estimating the stress on the mooring systems. The rainfall in Lake Nasser is only a few millimeters per year [21].
On the other hand, the average percentage of the sky covered by clouds experiences relatively small seasonal variation over the year. According to [22], the sky is mostly cloudy 25% of the time in December and clear, mostly clear, or partly cloudy 75% of the time. The clearer period of the year in Aswan is from mid-May to about mid-July. June is usually the clearest month of the year.
2.3. Water Temperature
In general, air and water temperatures increase from north to south [23]. The highest water temperature, 34.1°C, was recorded at Abu Simbel in summer; the lowest, 16.2°C, at the High Dam in winter [24]. The water temperature in the northern part of the lake is about 27°C in August, 22-23 degrees in November and March, and 16-17 degrees in February. The temperature also decreases with depth. At a depth of greater than 30 meters, the temperature remains between 16 and 20°C year-round.
Knowledge of water temperature is important in estimating the efficiency of the FPV. Satellite’s images were used to infer the surface water temperature [17]. It was found that the surface water temperature depends on the depth of water. A satellite image taken in March shows that in the middle of the lake (high depth area), the temperature varies between 12.4 and 18.9°C, in less depth near the shores it was found to be in the range of 18.9-21.5°C, while in shallow areas the surface water temperature was between 21.5 and 25.86°C.
Water Evaporation
The Egyptian quota from the Nile River is limited to be about 55 billion m 3 /y and is expected to decrease due to the increasing demand for water by other Nile basin countries [25]. According to an Egyptian government report [26], the total population of Egypt increased from 22 million in 1950 to around 85 million in 2010 (currently, it reached almost 100 million). The population is likely to reach between 120 and 150 million by 2050. Egypt has reached a state of water poverty where the available quantity of water is imposing limits on its national economic development [27]. As an indication of water shortage, the threshold value of 1000 m 3 /capita/year is usually used as an indicator. Egypt has already gone below this threshold since the nineties, and it is expected to cross the threshold of an absolute scarcity of 500 m 3 /capita/year by 2025 [26].
Egypt is stepping up efforts to address water scarcity at a high cost [28], pledging EGP 900 billion Egp (51 billion) for a national water management plan over 20 years.
In continuing efforts to fulfill its growing water needs, Egypt is set to build the largest seawater desalination plants in the world. The total existing desalination capacity is 235,600 m 3 /day. The new projects will add a total capacity of over one million m 3 /day. The installed cost of desalination plants is approximately 1m for every 1000 cubic meters per day of installed capacity.
On the other hand, the annual water losses by evaporation from Lake Nasser are estimated between 12 and 16 billion cubic meters [29], which is 20-30% of the Egyptian share of the Nile water. This water loss has been flagged as a serious national problem because the lake is the water bank of Egypt.
Estimation of the evaporation rate from Lake Nasser using Bowen ratio energy budget (BREB) method was proposed in [30]. Bowen ratio is the ratio between sensible heating and latent heating. Sensible heat transfer is connected with temperature difference while latent heat transfer is associated with change of states without change in temperature. The calculations were based on local meteorological and hydrological data from three floating weather stations.
The BREB evaporation rate is estimated using the following equation:
where is the evaporation rate in mm/day, is the density of the evaporating water, is the latent heat of evaporation (J/kg), is the specific heat of water, is the net radiation energy, is the Bowen ratio, is a
, is the reference temperature, is the lake surface temperature, is the increase in energy stored in the water body, and is the net energy carried into the water body because of inflow and outflow of water.
The BREB monthly average of evaporation rates ranged from 3.4 to 13.3 mm/day, with an overall average of 7.22 mm/day. This estimate comes close to the adopted figure of the Egyptian Ministry of Water Resources and Irrigation of 7.54 mm/day. The authors also compared the BREB method with results from six conventional evaporation quantification methods.
Another study in 2012 used twenty-four satellite images during the year 2008 to estimate the maximum and minimum evaporative losses from the lake at 16.3 and 12.5 billion cubic meters per year [31].
Hassan [32] estimated the rate of evaporation from Lake Nasser using the Surface Energy Balance Algorithm for Land (SEBAL). SEBAL is an image-processing model for calculation of the evapotranspiration (ET) rate based on satellite images of the spectral radiance in the near-infrared (NIR), visible (VIS), and thermal infrared (TIR) part of the spectrum [33]. The method was also adapted and applied for large water body in [34].
The surface energy balance equation is given by [32]
where is the net radiation (W/m 2 ) at the water surface. is estimated from Landsat TM spectral data of the lake. is the sensible heat flux, is the water mass evaporation flux, is latent heat of vaporization, is latent heat flux required for evaporation, is the increase in energy stored in the water body, and and are defined before. and were inferred from hydrological and meteorological measurement collected from three floating weather stations. Other meteorological data were also measured or inferred. The estimated evaporation rate was 6.6 mm/day [32].
A more recent study in 2018 [35] estimated the evaporation losses using two different techniques; the first method integrates Geographic Information System (GIS) techniques and MODIS images. The result showed that the water losses by evaporation from Lake Nasser ranged from about 12.3 to 12.9 billion m 3 per year. The second method found the annual losses to be 16.3 to 17.4 billion m 3 using data from MERRA images and ECMWF (ERA-Interim) [35].
Several studies proposed a variety of techniques to reduce the water lost by evaporation from Lake Nasser. A study in 2007 evaluated several methods for evaporation reduction from Lake Nasser, including floating cover sheets, similar to the one shown in Figure 7(a). The authors also proposed a new technique, where a pontoon framework is placed on the water surface, enclosing square surface areas of
filled with circular foam sheets, as depicted in Figure 7(b). Several options for the percent coverage and cover material were studied. They also estimated the yearly average evaporation rate to be 6.33 mm/day or about 2.3 m 3 per m 2 per year [13].
(a)
(b)
(a) (b)
(a) Example of commercial coversheets to prevent evaporation. (b) Proposed circular foam sheets [13].
In an effort to mitigate the evaporation losses from Lake Nasser, a study in 2010 proposed disconnecting (fully or partially) some of its secondary channels (Khors) [36].
In a recent study in 2019, the author examined two schemes to compensate for the water evaporation losses, namely, weather modification and water harvesting from the air. The study estimated the evaporation losses from Lake Nasser to be in the range from 5 to 10 mm/day throughout the year with an average of 7.4 mm/m 2 /day. This evaporation loss is estimated to be 20% of the annual consumption of water by Egypt for farming, industrial, and domestic applications [37].
A brief review of the existing techniques to reduce evaporation from water surfaces was conducted in 2019. The evaporation reduction techniques were classified as physical methods, biological methods, and chemical methods [38]. Physical methods, which include floating or suspended covers, can save a large percentage of water (between 70 and 95%); however, their capital costs are high. Biological methods, such as floating plants, windbreakers, and palm fronds, could provide a significant decrease in the volume of evaporation. However, the water drawn up and used for transpiration by plants needs to be taken into account to estimate the efficiency of using them for the purpose of evaporation reduction. over, concerns for long-term ecological impacts pose some restrictions on their uses.
Chemical methods, on the other hand, have relatively low capital cost (per m 2 of water surface). The fast and simple deployment methods of the chemical methods make them attractive for farm ponds and small lakes, but their effect depends on the surface condition including waves, wind, currents, and temperature. For large lakes, the maintenance costs of chemical methods could be significant, since under the action of currents, winds, and waves the chemical films can be displaced and destroyed [38].
The above evaporation studies consistently estimate the annual loss of water by evaporation from Lake Nasser to be at least 20% of Egyptian quota from the Nile River, and there is no viable solution to solve this problem.
On the other hand, it has been established in the literature that FPVs contribute to reduction of water loss by evaporation from the lakes [38–40]. For example, the study in [39] in Spain reported a theoretical and experimental analysis of covering a 4490 m 2 surface reservoir with floating PVs. The study reported annual water saving of 25% was achieved, while over 400 MWh of renewable power was generated per year. A review [40] of FPV technologies concluded that floating PV systems can reduce the evaporation up to 33% on natural lakes and ponds and 50% on human-made facilities.
The combined economic value of saving water and production of electricity renders the proposed project very attractive economically and reduces the breakeven time for the project.
Solar Energy Potential
Lake Nasser is located in one of the richest areas in solar energy in the world as shown in Figure 8 [41], with an average daily solar energy of about 6.72 kWh/m 2 Global Horizontal Irradiance (GHI), while the average Direct Normal Irradiance (DNI) reaches 7.92 kWh/day [42]. The yearly average DNI power is between 320 and 340 W/m 2. while the yearly average GHI power is between 280 and 290 W/m 2. The average PV potential in the Aswan region is estimated to be 2455 kWh/y per square meter [42].
However, the average daily incident solar energy experiences some seasonal variation over the course of the year. The brighter period of the year lasts for 3.9 months, from April 28 to August 25, with an average incident short wave energy of 7.6 kWh/day per square meter, with an average peak of 8.4 kWh/day per square meter. The darker period of the year, from November to January 31, has an average incident short wave energy per square meter of 5.3 kWh/day. The average monthly sun hours are close to 322 hours/month as shown in Figure 9. The average annual amount of sun hours is 3865.0 hours for 2018. The average in July and August exceeds 380 sun hours [43]. With a low-cost PV technology of 21% efficiency, we expect an average daily solar electric energy of 1.41 kWh/m 2.
In inland installations, the reflections from the surrounding hot land surface contribute to the overheating of land PVs and reduce the PV efficiency. The temperature has a negative effect on the PV cell power [44, 45]. A typical solar cell power versus temperature is shown in Figure 10. The figure shows a PV module peak power versus voltage when its temperature varies between 25°C and 65°C in steps of 20 degrees at a constant 1000 W/m 2 solar irradiance. The figure indicates clearly the negative effect of increased panel temperature on the panel output power.
Several studies verified the higher efficiency of FPV installations, between 10 and 17.5%, over inland installations due to the cooling effect of water bodies. For example, a study of the performance of 100 kW and 500 kW floating PV systems on a reservoir in South Korea showed that both systems provided a higher power generation during the whole test period compared to an overland PV system. The study concluded that the floating PV system has a higher production capacity by 7.6% to 13.5% than that of the ground-mounted PV system [46]. A modelling study showed that placing solar arrays on the water will increase their energy output and efficiency levels by 8% to 10% [47].
In spite of the hot air temperature, especially in summer, surface water temperature of Lake Nasser depicts mild temperature compared to the air temperature, as shown in Figure 11, and could considerably contribute to boost the efficiency of the FPVs.
Accordingly, based on the cited studies and the mild water temperature of Lake Nasser, we expect between 8 and 13% improvements in efficiency over inland installation operating under the same air temperature. However, further field studies are needed to obtain accurate figures to support the cost-benefit analysis of the operating companies.
Market and Economic Analysis
Europe’s consumption of electricity between 1990 and 2016 is shown in Figure 12. In 2016, Europe consumed almost 3100 TWh/y, which is about 8.49 Giga kWh/day [3].
Germany, France, and United Kingdom had the highest electricity generation in 2016 among the EU member states, with each double-digit share. According to Figure 13, the share of solar energy was just 3.5% of the total electric generation in 2016, while the combustible fuels account for 48.7%.
The Lake Nasser solar energy project is expected to be executed in two or three phases. The first phase is aimed at covering about 1000 km 2 of Lake Nasser by PV panels over a period of 10 years. Accordingly, the first phase of the project could supply enough electricity for Europe to replace at least 30% of combustible fuel in Europe, with the potential for entirely replacing combustible fuel by 2050, in the subsequent phases.
The study conducted by Fraunhofer ISE [5] forecasted the cost of wind, PV, and concentrated solar power (CSP) up to 2050 and concluded that by 2050 the electricity production costs for the generation potential of 2000 TWh/y will be €50/MWh for wind power, below 48€/MWh for PV, and under €56/MWh for CSP. According to this report, PV would be the lowest installation cost for massive projects and the lowest production cost. The report emphasised the importance of integrating the solar energy production in North Africa with the Europe power grid. Various economic studies also concluded that the PV solar electricity will be the lowest among all sources of electricity [48].
Another study by Fraunhofer ISE [49] indicated that the cost of electricity for industrial applications is between 7 and 18€/MWh among the European countries with an average of about 12€/MWh. On the other hand, the cost of household consumption is from 10 to 31/MWh and is expected to reach between 55 and 65€/MWh by 2040. The market size of the electricity sector in Europe is estimated to be 372 billion euros.
As such, the MENA solar electricity should reach the European market at 4-5 euro cents/kWh, including the transmission cost [50].
With the increasing CO2 taxes in Europe, the Egyptian solar electricity could easily penetrate the European electricity market, with the potential to overtake and replace the combustible fuel electricity market by 2050. The Lake Nasser solar energy project has the potential of meeting the European future needs of electricity, as the project continues to expand horizontally by increasing the coverage area and vertically by using more efficient PV technologies.
The first phase of the Lake Nasser project is aimed at covering about 20% of the Lake Nasser, which is almost 1000 km 2. The target surface area can be divided into approximately 25 km 2 lots. Egypt may then seek multi-international investors and contractors to build and operate this Tera project with revenue sharing for the lifetime of the project (20-25 years) or auction-based bidding, with a condition to remove the PV panels after the expiration of the license term.
First, we assume that about 20% of the assigned lot surface will be used for service. The actual covered surface area will then be 20 square km. We further assume the PV panels are horizontally laid and occupy about 0.8 of the floating platform. The remaining 20% of the platform surface is used for cables, power electronics, instrumentation, and walkways. The total PV surface
In this preliminary analysis, we assume that we use low-cost PV technology of 21% efficiency and the GHI of 6.72 kWh/m 2 at Aswan region [42]. The expected average daily solar electric energy will then be 1.41 kWh/m 2 per day.
The cost of a floating platform, if manufactured in Egypt, is estimated to be less than 1/m 2.
The last two (2019-2020) years have witnessed an accelerated decrease in the cost of PV installations. A review of the lowest solar power in the world, as of January 28, 2021, showed that the auction bidders’ price of kWh of utility scale PV installation around the word came down in the range from 1.35 to 1.97 cent/kWh [51]. This sharp drop in solar panels cost over the last few years came due to many advances in PV cell technology including the following: (i) Crystalline silicon (c-Si) PV cells are the most popular technology in PV installations, mainly because of its fast decrease in its cost (/Wp) and its proven reliability. As of 2020, monocrystalline silicon (c-Si) module pricing is around 0.20/W and multi-c-Si module pricing is around 0.17/W. However, it has limited efficiency and it is heavier than the thin film technology. Current research efforts FOCUS on ultrathin crystalline silicon absorber layers, improving production techniques and optimizing growth processes, which could lead to further decrease in its production cost and module weight [52] (ii) Concentrator photovoltaic (CPV) uses lenses or curved mirrors to FOCUS sunlight onto small, highly efficient, multijunction (MJ) solar cells. Experimental CPV modules demonstrated up to 46.0% efficiency. CPV could be promising technology for Lake Nasser installations because it works better under normal irradiation [53] (iii) Cadmium telluride (CdTe) panels are the most popular type of thin-film solar technology used in installations today. The main energy-producing layer is made from cadmium telluride. A substantial efficiency gain in the past several years, achieving lifetime efficiencies of 22–28%, is by implementing CdSeTe in the absorber layer [54]. over, the proposed CdSeTe technology improves lifetime and other important performance parameters of the solar cell [55] (iv) Passivated Emitter and Rear Cell (PERC) technology has significantly increased the efficiency of monocrystalline silicon (c-Si) and multi-c-Si modules and lowered their manufacturing cost. PERC technology increases the cell’s ability to catch longer wavelengths. The longer wavelengths are especially present during mornings and evenings or during cloudy days. Current, 2020, PERC PV panels have efficiency between 19 and 22% [56] (v) Other new high-efficiency technologies, but more costly, include IBC (interdigitated back contact cells) and HJT (heterojunction cells) [57]
These technological trends will continue to contribute to further reduction in the PV installation cost and/or increase the electricity production per m 2. Technology trends are also risk factors and must be examined carefully by the investors, and they need to weigh initial installation cost against long-term benefit from high-cost high-efficiency panels. (i) Disruptive technologies: during such a long-term project, Egypt needs to assess the risk and opportunities created by disruptive technology. For example, photocatalytic water splitting techniques to generate hydrogen have attracted great attention in the last a few years due to its potential for conversion and storage of solar energy [58–60]. Hydrogen has lucrative market (4-5/kg, 2020) for high-end cars, ammonia production, hydrogen cells, solar energy storage, petroleum refinery, in various industrial applications, and finally in recycling of CO2 to produce synthetic methane, methanol, and many hydrocarbon products [61]. As such, when this technology becomes mature for deployment over a large water area, it could be more profitable than FPV
Floating Platforms
The last few years have witnessed a significant growth of the FPV worldwide. The first large-scale float PV project in Europe was deployed in 2017, on the Queen Elizabeth II reservoir in the UK (Figure 14). The FPV development consists of 23,046 PV panels, each installed on a float and grouped together to form a floating platform. The solar panels were then towed to the middle of the reservoir and fixed in place using 177 anchors [62].
China completed in 2018 the world’s largest floating PV farm; see Figure 15. The project spread across 13 separate islets on an area of 140 hectares (1,400,000 m 2 ), with grid connection, testing, and commissioning carried out in March 2019 [63].
The Taiwanese government has embarked upon a 181 MW floating solar project at the Changhua Coastal Industrial Park. The project is the start of an ambition plan to install 20 GW of solar power by 2025 [64].
A floating solar PV system usually consists of five subsystems [65], as shown in Figure 16, the PV subsystem, the floating platform, the mooring subsystem, the underwater cables which transfer the generated power to the land, and the electric power and the control subsystem.
The floating subsystem includes the floating structure, pontoons, floaters, and mounting racks of the PV panels. The mooring subsystem stabilizes the position of the floating platform and automatically adjusts itself for the variation in the water level. A mooring system is made up of a mooring line and an anchor. The mooring line connects an anchor on the lake floor to the floating structure.
There are two main classes of design of the floating platforms: frame arrays and independent floatings [66]. The frame array is common in the over 1 MW installations. The system consists of rows of floats of cylindrical or rectangular section (see Figure 17), on which the structure of PV panels’ frames is arranged as repeated arrays. The system is easy to maintain and provides better ventilation and PV generation by water effect. Independent floatings are modular units, usually made of HDPE, which can be fixed together by connection points to form surface pontoons (Figure 18), on which the PV panels are mounted, using fiber-reinforced plastics (FRP) [67].
(a)
(b)
(a) (b)
The size of the floats is an important parameter in the design of the platform. The buoyant force, which is equal to the weight of displaced fluid, must be able to balance the gross weight of the platform under all operating and environmental conditions.
In the following example, we present a simplified calculation of the buoyant force for a platform module, which supports 8 standard 96-cell PV panels. The PV panels have frame dimensions of approximately.
Ex.1: the overall floating platform surface is
, supported by 3 high-density polyethylene (HDPE) closed-end pipes of diameter
, each of length 4.4 meters, as shown in Figure 17. The panels are mounted on galvanized steel racks. The weight budget is given in Table 2.
The parameters used for the following calculations are shown in Figure 19(b). The submerged pipe cross-sectional area. is given by
The buoyant force is given by
where is the total HDPE pipe length and is the density of the water.
(a)
(b)
(a) (b)
(a) Illustration of mounting racks carried by pipe pontoons. (b) Parameters used to calculate the bounce force in Ex.1.
Figure 20 shows the plot of the gross weight of the platform (to be carried by the buoyant force) and the submerged depth
The graph indicates that for the gross weight in Table 2 the submerged depth is about 21 cm.
The wide span of possible water level variation of Lake Nasser (about 6 meters every year) is a challenging problem and requires special considerations in the design of the anchoring and mooring system. Two common anchoring methods have been used for FPVs; anchoring at the banks is the most cost-effective anchoring system, while anchoring at the bottom is the most widely installed. Both options should be carefully studied.
To overcome the problem of varying water levels, anchoring isles can be placed at the corners of the floating platform, where anchoring wenches with automatic tension adjustment can be installed. Alternatively, a technique using hanging loads, called auxiliary mooring structure, is shown in Figure 21. The auxiliary mooring structure operates simply by gravity of the auxiliary weights.
A low-cost structure, shown in Figure 22, is being investigated at Zewail City of Science and Technology, Egypt, which utilizes UPVC pipes for the floating frame and marine-grade foam for the pontoons. The supporting structures of PVs are made of galvanized steel sections. The modules are made to fit inside standard shipping containers to facilitate transportations. The connection of the units is made with special rubber strips to mitigate the effect of 6D displacements between the units.
Considering PV tilting options, the flat horizontal panels could be the best option due to several reasons; being omnidirectional simplifies design and installation, eliminates shadow problems, and reduces maintenance cost. However, it is not theoretically efficient, compared with tilted solutions. Nevertheless, the loss of efficiency is compensated by the increase of efficiency due to the cooling effect of the water surface. The direction of the wind in Lake Nasser is mainly north (see Figure 6), which would make a flat design more favourable to avoid wind forces on the tilted structures.
The second tilting option is using a single tilt angle. However, it needs to be orientation-stabilized on the water surface, which increases the platform construction and installation cost and could create shadow problems and leaves limited options for modular design. On the other hand, it increases energy production by 5% over the flat platform. The fixed tilt angle at latitude of 22 degrees is about 18 degrees (facing south) [67].
For the two-angle option, Table 3, in addition to the increase in the cost of the platform modules, it substantially increases the maintenance and operational costs.
For four angles, the tilt angles for spring and fall would be about 20 degrees [68]. Fully steerable 2D PVs need lifetime cost-benefit analysis, considering the potential for increased output by 25% over the flat surface. The use of PV tilting options requires new innovative solutions to be a truly viable option in such massive projects.
Solar Energy Storage Solutions
Solar energy storage enables the use of solar energy 24 hours. With the fast introduction of solar energy, the energy storage market will see Rapid expansion in the next few years: the global storage market is forecasted to reach at least 250 billion by 2040 [69].
Expanding the use of solar energy requires, on the one hand, an hourly based tariff to encourage customers to save solar energy during the day and, on the second hand, a distributed approach for solar energy storage. The geographically distributed solution could be at three vertical levels of energy storage: (1) Residential battery storage (2) Industry/enterprise level energy storage (3) Utility or state energy storage
The residential energy storage batteries can be made to charge when tariff is the lowest and turned on during the highest tariff rates. Such small-scale electric storage solutions will have a crucial role in expanding onsite consumption. There are several emerging battery technologies which promise low cost and higher capacities [70].
For industry and enterprises with up to 200 MW, cryogenic energy storage [71] could be a very promising solution. Cryogenic technology converts air to liquid by cooling down air to.196°C (-320°F). The air then can be efficiently stored in insulated rooms. When air is brought to ambient temperatures, it regasifies and its volume expands to 700-fold, which is then used to drive a turbine and generate electricity without combustion. These systems can be built from 10 MW to more than 200 MW power output, with a storage capacity more than 2000 MWh. The lifespan of cryogenic energy storage systems is over 30 years with efficiency between 60 and 75%. Large-scale batteries are also available [72] for small and medium enterprises.
For utility and large-scale energy storage, two technologies are available; the first one is hydraulic pumping, where pumps are used to move water to higher level tanks, converting the electric energy to gravitational energy during the day time and then using it to drive turbine generators at night. Because of the simplicity and low cost of this approach, pumped hydro plants account for almost 96% of current large-scale installations [70, 73]. In addition, pumped hydro plants are a mature technology, capable of storing huge amounts of energy, and have relatively high efficiency (70-80%) and expected lifespan of 40-60 years. Pumped hydro systems could be a viable energy storage solution bringing water from Lake Nasser to nearby higher level areas, which exist on the east side of Lake Nasser. Nevertheless, such hydraulic plants are expected to support only a few gigawatts of power, which is far below the scale of the project. We believe a distributed energy storage solution would be the most economical and practical solution. The second approach for utility scale energy storage is to convert energy into fuel, for example, using electricity to generate H2 from water by electrolysis [73, 74]. Hydrogen may then be stored or transported and used as a fuel. The generated H2 could be used in a process known as methanation [74], where the generated H2 is combined with captured CO2 to generate synthetic methane, which could then be stored and transported. The process has a positive impact on the environment by utilizing captured CO2 from CO2-emitting industries.
Impact on Environment and Fishery Sector
The current production of fish from Lake Nasser is about 26,000 ton/y that is less than 5.2 ton per/km 2 or 5.2 kg/1000 m 2. In fact, it is reported that during the recent years, with the growing number of fishing boats and poor compliance with the technical measures, the yield from the fishery in Lake Nasser has exhibited a declining trend since 1981, indicating unsustainable rates of exploitation [75].
On the other hand, according to [76], cage fish farming along a coastal line could yield 20 kg per m 3 of water with trained operators and properly regulated and managed cage farming [77].
The large number of small Khors along the coastline of Lake Nasser can be converted to fish enclosures [78] by closing their entrance by a large net. Enclosures increase production per unit of area and make it possible to adopt intensive culture practices and protection from predators. As such, the impact of the project on Lake Nasser fishery can be mitigated by modernizing the current unsustainable fishing practices. over, the available low-cost electricity from the project will enable the introduction of state-of-the-art value chain and introduction of lucrative fish industries.
On the other hand, several positive environmental impacts of FPVs were consistently reported in the literature. For example, it is reported in [79] that the shade provided by the FPVs reduces the presence of algae blooms in bodies of freshwater. Algae blooms can be dangerous for human health if they occur in source of drinking water and can also lead to the death of plants and animals living in the body of water.
An NREL-sponsored study [80] in the USA reported that floating PVs can help mitigate algae growth in water bodies. Another benefit of shading water surfaces is the reduction of the incidence of solar radiation in water and therefore its temperature. The reduction in water temperature increases phytoplankton growth rates, which is an important player in the fish food chain [81].
During the course of execution and operation of such a massive project, several precautions must be taken into consideration to avoid adverse impacts on the environment. In a study sponsored by the Asian Development Bank (ADB) [82], it discusses several potential environmental impacts of FPV projects and proposes a number of mitigation measures. The construction phase usually involves site preparation (e.g., grading and levelling), transportation, installation, and commissioning of infrastructure (including the floats, PV panels, inverters, transformers, access road, transmission lines, and land control centres). These activities are likely to generate air emissions and impacts on land and aquatic habitats. Mitigating measures include land and water traffic management, and regulatory measures during the construction phase should be enforced regarding using appropriate machinery, trucks, cars, and waste and oil waste disposal measures.
During operation, it is also recommended to place zoning, water and landmarks, and separation of the regular waterway traffic from the maintenance traffic. Contractors will be responsible to report and clean construction wastes, debris, and parts that could accidentally fall in the land or in water. In addition, the use of chemicals on the floating PVs should be prohibited.
Finally, the license contract should stipulate removal the PV panels at the end of the project and dispose them in accordance with the recommendations of the International Renewable Energy Agency (IRENA) and the International Energy Agency Photovoltaic Power Systems (IEA-PVPS) [83] or according to the international regulations at the time of the decommissioning of the project.
Nevertheless, the project is expected to generate a range of positive impacts on the local community including local training and employment opportunities as well as substantial growth opportunities for local business and industry.
Conclusions
The paper presented a study of the energy production and water saving of a massive deployment of floating PVs over Lake Nasser in south Egypt. The area is very rich in solar energy. It also enjoys mild wind, low surface waves, and almost year-round sunshine. If only 20% of the lake is covered, about 1000 km 2. it will produce low-cost green electricity that is enough for almost 16% of European need, with the potential to entirely replace coal- and fossil-based electricity in Europe during the subsequent phases of the project. The project will also help to reduce water loss by evaporation from Lake Nasser, which represents almost 20-25% of the Egyptian yearly consumption of water. The availability of low-cost electrical energy could make Lake Nasser’s neighbourhood a world hub for electric energy intensive industries.
Data Availability
Links to the sources of data are listed in the reference section. Generated data and calculations will be made available after publication, upon request.
Conflicts of Interest
The authors declare that there is no conflict of interest regarding the publication of this paper.
Acknowledgments
The authors would like to thank Zewail City of Science and Technology for its support of this study.
References
J. Vogler, “The European contribution to global environmental governance,” International Affairs, vol. 81, no. 4, pp. 835–850, 2005. View at: Publisher Site | Google Scholar
T. Buck, “Decline in new German wind farms sparks concern,” Financial Times, 2019, https://www.ft.com/content/b0f94478-c998-11e9-a1f4-3669401ba76f. View at: Google Scholar
W. Platzer, I. Boie, M. Ragwitz et al., Supergrid study– approach for the integration of renewable energy in Europe and North Africa, Technical report, Fraunhofer Institute for Solar Energy Systems ISE, 2016.
F. Haugwitz, “Floating solar PV gains global momentum,” PV Magazine, September, vol. 22, 2020, https://www.pv-magazine.com/2020/09/22/floating-solar-pv-gains-global-momentum/. View at: Google Scholar
World Bank Group, ESMAP and SERIS, Where Sun Meets Water: Floating Solar Market Report, World Bank, Washington, DC, 2019. View at: Publisher Site
R. Cazzaniga and M. Rosa-Clot, “The booming of floating PV,” Solar Energy, vol. 6, 2020. View at: Publisher Site | Google Scholar
R. M. A. Hassan, N. T. H. Hekal, and N. M. S. Mansor, “Evaporation reduction from Lake Naser using new environmentally safe techniques,” in Eleventh International Water Technology Conference, IWTC11 2007 Sharm El-Sheikh, Egypt, 2007. View at: Google Scholar
M. A. El-Shirbeny and K. A. Abutaleb, “Monitoring of water-level fluctuation of Lake Nasser using altimetry satellite data,” Earth Systems and Environment, vol. 2, no. 2, pp. 367–375, 2018. View at: Publisher Site | Google Scholar
E. Muala, Y. A. Mohamed, Z. Duan, and P. van der Zaag, “Estimation of reservoir discharges from Lake Nasser and Roseires Reservoir in the Nile Basin using satellite altimetry and imagery data,” Remote Sensing, vol. 6, no. 8, pp. 7522–7545, 2014. View at: Publisher Site | Google Scholar
H. M. Ebaid and M. Aziz, “Integrating radar altimeters and optical imagery data for estimating water volume variations in lakes and reservoirs (case study: Lake Nasser),” Journal of Geographic Information System, vol. 9, no. 6, pp. 648–662, 2017. View at: Publisher Site | Google Scholar
M. M. Mostafa and H. K. Soussa, “Monitoring of Lake Nasser using remote sensing and GIS techniques,” in ISPRS Mid-term Symposium Proceeding, May 2006. View at: Google Scholar
M. I. El-Mekawy, Z. Salah, and M. M. A. Wahab, “Climatology of Lake Nasser in Egypt,” Middle East Journal of Applied Sciences, vol. 8, no. 3, pp. 719–726, 2018. View at: Google Scholar
R. H. Goma, “Seasonal and spatial variation of nutrients,” in Sustainable fish production in Lake Nasser: ecological basis and management policy, J. F. Craig, Ed., ICLARM Conf. Proc, pp. 33–38, International Center for Living Aquatic Resources Management (ICLARM), Penang, Malaysia, 2000. View at: Google Scholar
G. M. El-Shabrawy, “Lake Nasser—Nubia,” in The Nile. Monographiae Biologicae, H. J. Dumont, Ed., vol. 89, Springer, Dordrecht, 2009. View at: Publisher Site | Google Scholar
Water politics. sharing the Nile, The Economist, Middle East and Africa, 2016, December 2019, http://www.economist.com/middle-east-and-africa/2016/01/16/sharing-the-nile.
M. E. D. M. Omar and A. M. A. Moussa, “Water management in Egypt for facing the future challenges,” Journal of Advanced Research, vol. 7, no. 3, pp. 403–412, 2016. View at: Publisher Site | Google Scholar
M. A. Mosalam Shaltout and T. El Housry, “Estimating the evaporation over Nasser Lake in the upper Egypt from meteosat observations,” Advances in Space Research, vol. 19, no. 3, pp. 515–518, 1997. View at: Publisher Site | Google Scholar
M. Elsawwaf, P. Willems, A. Pagano, and J. Berlamont, “Evaporation estimates from Nasser Lake, Egypt, based on three floating station data and Bowen ratio energy budget,” Theoretical and Applied Climatology, vol. 100, pp. 439–465, 2009. View at: Publisher Site | Google Scholar
I. H. Abou El-Magd and E. M. Ali, “Estimation of the evaporative losses from Lake Nasser, Egypt using optical satellite imagery,” International Journal of Digital Earth, vol. 5, no. 2, pp. 133–146, 2012. View at: Publisher Site | Google Scholar
M. Hassan, “Evaporation estimation for Lake Nasser based on remote sensing technology,” Ain Shams Engineering Journal, vol. 4, pp. 593–604, 2013. View at: Publisher Site | Google Scholar
W. G. M. Bastiaanssen, M. Menenti, R. A. Feddes, and A. A. M. Holtslag, “A remote sensing surface energy balance algorithm for land (SEBAL) 1. Formulation,” Journal of Hydrology, vol. 212–213, pp. 198–212, 1998. View at: Publisher Site | Google Scholar
A. Ashfaque and W. G. M. Bastiaanssen, “Estimating evaporation from Lake Naivasha, Kenya using remotely sensed Landsat Thematic Mapper (TM) spectral data,” Journal of Civil Engineering, The Institution of Engineers, Bangladesh, vol. CE28-2, 2000. View at: Google Scholar
M. M. Abdel Wahab, Y. H. Essa, A. A. Khalil, K. Elfadli, and G. Panegrossi, “Water loss in Egypt based on the Lake Nasser evaporation and agricultural evapotranspiration,” Environment Asia, vol. 11, pp. 192–204, 2018. View at: Google Scholar
H. M. I. Ebaid and S. S. Ismail, “Lake Nasser evaporation reduction study,” Journal of Advanced Research, vol. 1, no. 4, pp. 315–322, 2010. View at: Publisher Site | Google Scholar
B. E. Abulnaga, “Harvesting the skies of Egypt: an option to recover the evaporation losses from the Aswan High Dam Reservoir,” in Grand Ethiopian Renaissance Dam Versus Aswan High Dam. The Handbook of Environmental Chemistry, A. Negm and S. Abdel-Fattah, Eds., vol. 79, pp. 385–415, Springer, Cham, 2019. View at: Publisher Site | Google Scholar
Y. W. Youssef and A. Khodzinskaya, “A review of evaporation reduction methods from water surfaces,” E3S Web of Conferences, vol. 97, article 05044, 2019. View at: Publisher Site | Google Scholar
M. R. Santafé, J. B. T. Soler, F. J. S. Romero, P. S. F. Gisbert, J. J. F. Gozálvez, and C. M. F. Gisbert, “Theoretical and experimental analysis of a floating photovoltaic cover for water irrigation reservoirs,” Energy, vol. 67, pp. 246–255, 2014. View at: Publisher Site | Google Scholar
A. Sahu, N. Yadav, and K. Sudhaka, “Floating photovoltaic power plant: a review,” Renewable and Sustainable Energy Reviews, vol. 66, pp. 815–824, 2016. View at: Publisher Site | Google Scholar
“Solar resource maps and GIS data for 180 countries, Solargis,” December 2020, https://solargis.com/maps-and-gis-data/download/egypt. View at: Google Scholar
P. Kosmopoulos, S. Kazadzis, and H. El-Askary, Solar Atlas of Egypt, European Horizon 2020 Project# NO 690133, 2018.
“Average monthly hours of sunshine in Aswan (Aswan Governorate), Egypt,” December 2020, https://weather-and-climate.com/average-monthly-hours-Sunshine,Aswan,Egyp. View at: Google Scholar
A. Razak, Y. M. Irwan, W. Z. Leow, M. Irwanto, I. Safwati, and M. Zhafarina, “Investigation of the effect temperature on photovoltaic (PV) panel output performance,” International Journal on Advanced Science, Engineering and Information Technology, vol. 6, pp. 682–688, 2016. View at: Publisher Site | Google Scholar
S. Dubey, J. N. Sarvaiya, and B. Seshadri, “Temperature dependent photovoltaic (PV) efficiency and its effect on PV production in the world. a review,” Energy Procedia, vol. 33, pp. 311–321, 2013. View at: Publisher Site | Google Scholar
Y. K. A. Choi, “Study on power generation analysis of floating PV system considering environmental impact,” International Journal of Software Engineering and Its Applications, vol. 8, pp. 75–84, 2014. View at: Publisher Site | Google Scholar
A. McKay, Floatovoltaics: quantifying the benefits of a hydro-solar power fusion, [Pomona Sr. theses], Claremont University, 2013.
P. Ralon, Global renewable energy cost trends, International Renewable Energy Agency IRENA, 2018, http://www.reeem.org/wp-content/uploads/2018/04/Pablo-Ralon-IRENA.pdf.
C. Kost, S. Shammugam, V. Jülch, H. T. Nguyen, and T. Schlegl, Levelized cost of electricity renewable energy technologies, Fraunghofer ISE, 2018.
A. Dobbins, F. Fuso Nerini, P. Deane, and S. Pye, “Strengthening the EU response to energy poverty,” Nature Energy, vol. 4, no. 1, pp. 2–5, 2019. View at: Publisher Site | Google Scholar
D. F. R. Margolis, Q2/Q3 2020 Solar industry update, National Renewable Energy Laboratory (NREL), US, 2020, https://www.nrel.gov/docs/fy21osti/78625.pdf.
M. Steiner, T. Gerstmaier, and A. W. Bett, “10. Concentrating photovoltaic systems,” in The Performance of Photovoltaic (PV) Systems, N. Pearsall, Ed., pp. 297–320, Woodhead Publishing, 2017. View at: Publisher Site | Google Scholar
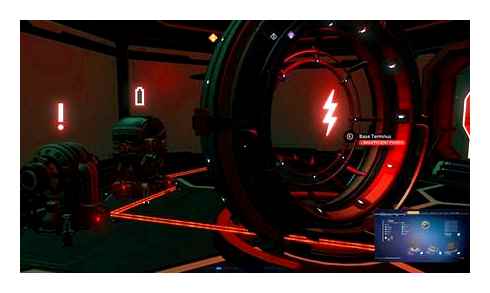
W. K. Metzger, S. Grover, D. Lu et al., “Exceeding 20% efficiency with in situ group V doping in polycrystalline CdTe solar cells,” Nature Energy, vol. 4, no. 10, pp. 837–845, 2019. View at: Publisher Site | Google Scholar
M. Rong Wang, “Enhanced performance of Se-alloyed CdTe solar cells: the role of Se-segregation on the grain boundaries,” Journal of Applied Physics, vol. 129, no. 2, article 024501, 2021. View at: Publisher Site | Google Scholar
A. Blakers, “Development of the PERC solar cell,” IEEE Journal of Photovoltaics, vol. 9, no. 3, pp. 629–635, 2019. View at: Publisher Site | Google Scholar
C. Y. Toe, Z. Zheng, H. Wu, J. Scott, R. Amal, and Y. H. Ng, “Transformation of cuprous oxide into hollow copper sulfide cubes for photocatalytic hydrogen generation,” The Journal of Physical Chemistry C, vol. 122, no. 25, pp. 14072–14081, 2018. View at: Publisher Site | Google Scholar
H. Wu, “A pulse electrodeposited amorphous tunnel layer stabilises Cu2O for efficient photoelectrochemical water splitting under visible-light irradiation,” Journal of Materials Chemistry A, vol. 8, no. 11, pp. 5638–5646, 2020. View at: Publisher Site | Google Scholar
M. Wang and G. Lu, “Improved light harvesting and efficiency for overall water splitting by embedding TiO2 transition,” RRL Solar, Wiley Online Library, 2020. View at: Publisher Site | Google Scholar
E. C. Ra, K. Y. Kim, E. H. Kim, H. Lee, K. An, and J. S. Lee, “Recycling carbon dioxide through catalytic hydrogenation: recent key developments and perspectives,” ACS Catalysis, vol. 10, no. 19, pp. 11318–11345, 2020. View at: Publisher Site | Google Scholar
“Europe’s largest floating solar farm, Lightsource BP,” December 2020, https://www.lightsourcebp.com/uk/stories/qe2/. View at: Google Scholar
S. H. Kim, S. J. Yoon, and W. C. Choi, “Design and construction of 1 MW class floating PV generation structural system using FRP members,” Energies, vol. 10, no. 8, article 1142, 2017. View at: Publisher Site | Google Scholar
“Solar Electricity Handbook, 2019 Edition, Solar Panel Angle Calculator,” December 2020, http://www.solarelectricityhandbook.com/solar-angle-calculator.html. View at: Google Scholar
T. M. Gur, “Review of electrical energy storage technologies, materials and systems: challenges and prospects for large-scale grid storage,” Energy Environmental Science, vol. 11, pp. 2696–2767, 2018. View at: Publisher Site | Google Scholar
A. Poullikkas, “A comparative overview of large-scale battery systems for electricity storage,” Renewable and Sustainable Energy Reviews, vol. 27, pp. 778–788, 2013. View at: Publisher Site | Google Scholar
O. Achkari and A. El Fadar, “Renewable energy storage technologies. a review,” in Conference Internationale en Automatique Traitement de Signal (ATS-2018), Proceedings of Engineering and Technology –PET, vol. 35, pp. 69–79, 2018. View at: Google Scholar
J. Gao, Y. Wang, Y. Ping et al., “A thermodynamic analysis of methanation reactions of carbon oxides for the production of synthetic natural gas,” RSC Advances, vol. 2, no. 6, pp. 2358–2368, 2012. View at: Publisher Site | Google Scholar
A. Halls, “Lake Nasser fisheries: recommendations for management, including monitoring and stock assessment,” Tech. Rep., Penang, Malaysia, WorldFish Program Report, 2015. View at: Google Scholar
Food and Agriculture Organization of the United Nations, 4. Aquaculture methods and practices: a selected review, FAO, December 2020, http://www.fao.org/3/t8598e/t8598e05.htm.
J. M. Njiru, C. M. Aura, and J. K. Okechi, “Cage fish culture in Lake Victoria: a boom or a disaster in waiting?” Fisheries Management and Ecology, vol. 26, pp. 426–434, 2018. View at: Google Scholar
O. A. Habib, M. Shehata, M. Zaki, H. Ammar, and S. H. Abdel Rahman, “Building fish enclosure in Lake Nasser,” in Lake Nasser Development Authority, WorldFish Center technical manual no.1950, The WorldFish Center, Penang, Malaysia, 2009. View at: Google Scholar
R. S. Spencer, J. Macknick, A. Aznar, A. Warren, and M. O. Reese, “Floating photovoltaic systems: assessing the technical potential of photovoltaic systems on man-made water bodies in the continental United States,” Environmental Science Technology, vol. 53, pp. 1680–1689, 2019. View at: Publisher Site | Google Scholar
M. A. E. Galdino and M. M. de Almeida Olivieri, “Some remarks about the deployment of floating PV systems in Brazil,” Journal of Electrical Engineering, vol. 5, pp. 10–19, 2017. View at: Publisher Site | Google Scholar
Asian Development Bank (ADB), “Floating solar energy project. Initial environmental and social examination report,” in Project Number: 51327-001, Environmental Resources Management (ERM), Vietnam, 2018. View at: Google Scholar
S. Weckend, A. Wade, and G. Heath, “End-of-life management: solar photovoltaic panels,” Tech. Rep., International Renewable Energy Agency (IRENA) and the International Energy Agency (IEA), Report Number: T12-06:2016, 2016. View at: Google Scholar
Copyright
Copyright © 2021 Moustafa Elshafei et al. This is an open access article distributed under the Creative Commons Attribution License, which permits unrestricted use, distribution, and reproduction in any medium, provided the original work is properly cited.
How to get unlimited electricity for your base in No Man’s Sky (2022)
No Man’s Sky is a space exploration game in its truest sense, as it allows players not only to roam across numerous galaxies but to settle on a single planet by building a home. Everyone can choose their terrain, build their structure, and start a new life, even as a farmer, with the number of choices No Man’s Sky throws at them.
However, most basic structures require source of electricity to get up and run. While the most basic way to gather power on any planet is via a Battery and a Solar Panel, things tend to feel pretty limited if you have a big picture in mind. Thankfully, there is a way to get an unlimited source of power for your base.
Guide to getting unlimited power for your base in No Man’s Sky (2022)
Step 1
To get started, you need to have your Anomaly unlocked. It appears in space after you have made one warp to a different Galaxy. Once you’re cleared, open your in-game menu and summon the Anomaly.
Once you’re in, go up the stairs and head far back to where the upgrade merchants are. Go to the Multi-Tool upgrade merchant on the far right and purchase the Survey Device upgrade with your Nanites. It requires 3 Magnetic Resonators, 1 Quantum Computer, and 2 Wiring Looms.
Most of these items can be bought through a terminal on any Space Station.
Step 2
After installing your Survey Device, you need to use it on any given planet. Once you’re done choosing a planet you’d like to build your base on, open your Analysis Visor and switch to Power Survey Mode.
If the screen shows you something like Hotspot Proximity, followed by a specific number of distances, you now have a location where you can get infinite electricity. If nothing shows up on your Survey Device, try going further from your current location and looking for it again.
Step 3
Once you have found your Hotspot, go back to Anomaly and look for the Electromagnetic Generator blueprint in the Construction Module panel. It requires 2 Metal Platings, 60 Magnetized Ferrite, and 75 Chromatic Metals. Once crafted, try placing it near the center of the Hotspot.
Depending on the range from the core, it will supply power for your entire base. Each Electromagnetic Generator has four outlets from which you can start wiring your bases and gadgets. You can even place multiple Generators to stack the overall power supply.
As long as each Generator stays within the radius of the main Hotspot, you can place more than one. However, wiring each gadget from the Generator needs to be done within a particular range.
All you need to know to power your home with solar
Solar panels generally have a life of 25 years so they may be lighting up your toddler’s wedding functions.
Did the world turn black when you saw your latest electricity bill? If so, you are not alone.
The heat demands air conditioning, electricity providers demand fistfuls of money, the International Monetary Fund demands higher tariffs and Russian President Vladimir Putin demands Ukraine’s annihilation.
But it is impossible to explain the concoction of reasons to a toddler who just wants to sleep with a teddy bear while the AC is running. Harnessing solar power is a happy solution for those who can afford it.
Here are some factors to consider before opting for a solar solution:
Quality
One can take a gander down Regal Chowk in Karachi and pick up a solution that costs a few hundred thousand rupees compared to a million-plus option that a branded supplier could provide but it comes with its set of drawbacks.
The solution providers at the lower price end tend to import a container of solar paraphernalia and hire an everyday electrician to install it. Given its technical nature, the lack of support may create some issues.
Shoddy-quality solar panels can cause short circuits and sparks. In the best-case scenario, the system will shut down and you will be chasing after the supplier that may or may not be able to provide after-sales service.
“Even if a top-tier branded solar solution was installed in your house, it can act up a year or two after purchase,” says Musa Khan Durrani, the head of business and planning at SkyElectric. “You call up your local supplier but he may have switched his business to real estate and suggests you contact the manufacturer directly.”
“In the worst-case scenario, the sparks from inferior quality panels can start a fire,” cautions Mujtaba Raza, CEO of Solar Citizen.
Registration
Reputable solar providers are registered with the Alternative Energy Development Board (AEDB). While AEDB in no way vets or guarantees the quality of the panels or service, its registration allows the supplier to set up the net metering system which is where all the cost savings lie.
Compare it to buying a car: given the price tag and longevity of the purchase, you would opt for a trusted name such as Toyota or Honda rather than an unknown entity, if your budget permits. Solar panels generally have a warranty of 10-15 years and a life of 25 years so they may be lighting up your toddler’s wedding functions.
Net metering
Cost savings lie in net metering, which is a billing mechanism that credits solar energy system owners for the electricity they add to the grid.
A company registered with AEDB can set up the net metering process on behalf of its customer. According to its website, KE processes applications received by AEDB-listed vendors only though this may not apply to all electric supply companies.
For example, you have a bungalow in Defence, Karachi, with a photo voltaic system on your terrace. It may generate more electricity than you need during the day, allowing you to sell the surplus to K-Electric.
Using the numbers of your annual electricity consumption, some companies can offer you a package that will bring down your electricity bill to zero. If your household consumes Rs1,000 of electricity at night on average, you can sell Rs1,000 worth of credits while the sun shines that can be availed after the sun goes down.
So during the day, you would use your own generated solar energy and KE would purchase the excess. Without batteries, at night, you would shift back to using electricity by KE which means load shedding at night requires the use of the good old generator running on the ever-pricey diesel.
To avoid power outages completely, you will need batteries to store the electricity generated while the sun is shining. There are different kinds of batteries, from the tubular batteries you are familiar with from the UPS system to the more expensive solar-specific solutions that can cost a further Rs250,000 per kilowatt for a home solution from a reputable company.
Solar solutions are less feasible for apartments because there is not enough open space that gets direct sun exposure. Though arguably, common areas such as parking can theoretically be solarised which may not take a complete load of all the apartments but can subsidise electricity bills.
Upfront cost and maintenance
Ali Karimjee, a resident of DHA in Karachi, installed an 11kW solar system in 2018 that cost him about Rs1.1m. “With the credits I earn through solar, I don’t pay electricity bills during the cooler months from November to March,” he says.
At the previously lower tariffs, at the peak of summer, his bill did not exceed Rs7,000-8,000 per month though three air conditioners, two fridges and an assortment of household appliances run on electricity. As electricity tariffs climb, he expects higher savings.
All this is peachy keen but the cost of solar is a hefty one and rises with the exchange rate. “As a rule of thumb, a normal household with three to four ACs and the usual appliances requires a solution of 10kW which now costs roughly Rs1.3m without batteries,” says Raza.
However, it can go as high as Rs2m, says Durrani. A 10kW system, including batteries, can cost between Rs1.8m to Rs3m but while the panels can last decades, the batteries may have a life of only a couple of years.
Being completely independent from grid electricity entirely is not the wisest of options. Batteries are expensive and need to be replaced frequently, so it could be upwards of an extra Rs300,000 every two years. If the purpose of the PV installation is to decrease the electricity bill, then a hybrid solution adds up to the most savings. If the purpose is to thumb your nose at KE, than by own means, go ahead.
A small additional cost is related to maintenance which involves washing the solar panels frequently. “A man comes to service my solar panels every week,” says Karimjee. “I pay him Rs3,500 per month.”
Financing
How can one afford a Rs1m-plus solar system? The State Bank offers financing at a flat six per cent rate, which is not linked to the Karachi Interbank Offered Rate. Generally, the tenure of the loan is three to five years with a down payment of 20pc equity.
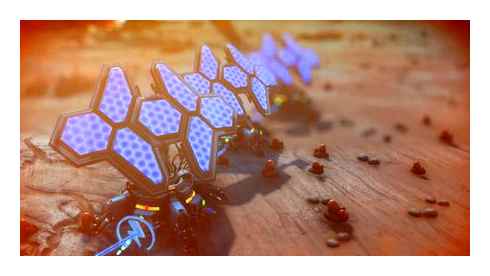
Depending on electricity consumption and the amount spent on the solar solution, what you owe the bank could tally up to a smaller figure at the end of the month compared to your bill. For example, if you spend Rs25,000 a month paying off the bank when your electricity consumption is at Rs40,000, you will be saving money, even with a loan.
Keep in mind that while electricity tariffs increase, the amount of instalments remains constant so your savings will increase in proportion to the rise in energy rates.
However, banks take months to process solar loans, industry insiders complain. Given the technology involved, panels and assorted parts are imported and the Rapid depreciation of the rupee jacks up prices. A few months down the road when the loan is finally processed, the cost of the package could have risen as well.
Solar Panel To Battery Ratio (Kw Watts)
Designing a home solar energy system is certainly an exciting process, but it can become rather confusing too. When designing your system, there are two vital components to consider first; solar panels and batteries.
Once you’ve decided your energy needs, you’ll need to decide how many batteries you need and what size panels are required to charge your battery bank.
However, this is easier said than done, and just what is the correct solar panel to battery ratio?
In general, you’ll need a panel that can charge your batteries at a steady rate so that you can have them fully or almost fully charged at the end of the day. This charge rate depends on a variety of factors, but there are some formulas to help you choose the perfect panel/battery ratio.
In this article, we’ll be covering the following:
- Choosing the right panel/battery combination
- Calculating panel to battery ratios
- Calculating your panel and battery power needs
If you’ve just invested in a new battery for your solar system and want to know what panel you need to run it properly, you’ve come to the right place!
How to choose a battery for a solar panel?
Choosing the right panel and battery combination depends on a variety of factors, including:
- Your energy consumption. How much power are you currently using every day?
- Your location. Do you live close to the equator? How much sun do you get every day, and how much-overcast weather is there in your area?
- Your energy needs. What are the energy needs of your solar setup? Are just using it for backup or are you completely off-grid?
- Your budget. While solar panels and other solar products have become more affordable in the past decade, converting to 100% solar power is still not cheap. Your budget may dictate how quickly you can make the transition.
When matching your panel and battery, consider the above points before making a decision, as this will help you make the best choices for your system in the long run. Let’s look at how to choose the battery for a solar panel.
A good general rule of thumb for most applications is a 1:1 ratio of batteries and watts, or slightly more if you live near the poles.
For example, if you have a 100-watt panel producing about 6 amps per hour, or 30aH per day, coupled with a 200aH battery, your battery will not be getting enough amps for it to charge fully within a day or two, unless you are using less than 30ah per day.
This could work if you are only drawing minimal power for charging phones or something similar, but it will put unnecessary strain on your solar setup.
Alternatively, a 300-watt panel charging a 100aH battery results in much wastage.
Even if you were using the battery to its maximum potential daily, you would only need 50aH of incoming charge per day to charge the battery fully. A 300-watt panel can easily provide double that, which is not used to its full potential.
As we mentioned earlier, a bigger panel-to-battery ratio is preferable in areas where you are not getting very much sun or if you live closer to the poles.
Ideally, no matter your application, the 1:1 ratio is a good rule to follow, especially for small solar setups under a kilowatt. A 100-watt panel and 100aH battery is an ideal small setup; you can expand it from there.
Matching solar panel to battery size
Let’s take a look at the general rule of thumb mentioned earlier: a 1:1 ratio of batteries and watts. A 200-watt panel and 200aH battery is a great combination to begin with.
If you’re using a 200-watt solar panel you can estimate roughly 15 amps of incoming power per hour — in perfect conditions.
This will equate to roughly 7 hours of charge time, or 100aH per day, depending on where you live and how much sun reaches your panel.
It’s important to note that a 200aH battery will only have 100aH (50%) of usable power, as going any lower than this will potentially ruin your battery, or dramatically shorten its life in the best-case scenario.
With a 200aH battery and a 200-watt panel, you should be able to fully charge your battery — or at least get very close — in a single day.
With this formula in mind, you’ll need to calculate your energy needs, and then from there, you can estimate what battery storage you need, and then what panel you’ll need to charge the batteries sufficiently.
How do you calculate a battery for a solar panel?
There is a simple formula for deducing what panel size you need for your battery, but this depends on how many hours of sunlight(roughly) you’re getting per day, which, for most cases, we can average out at around six. This simple formula is:
Battery amp hours are multiplied by voltage and then divided by daylight hours.
For example, a 100ah, 12v battery would be 100×12=1,200. This divided by 6 hours equals 200. So, if you want to charge a 100ah battery from flat to full daily, a 200-watt panel in ideal conditions would do it.
Now that we’ve got a better idea of what to consider when matching a solar panel and batteries, let’s take a look at the best panel size for particular battery setups.
Ideally, you’ll want slightly more power coming from your panel than you need from your batteries, as this will reduce the chance of running out of power and help during overcast days.
Remember, these are rough estimates, like your location and your energy needs will also play a part in the battery and panel size you need. Here are the best panel sizes — in general — for most common battery specifications.
12v battery
A 12v battery needs at least 13.6 volts to charge efficiently. However, a 12v battery can be as small as 50aH or as big as 200aH, so the amp hour rating of your battery is most important.
With that said, you’ll need a panel that is delivering between 13.6 and 17 volts, and depending on your battery’s ah rating and your power needs, we recommend a panel of at least 100 watts.
24v battery
Panels made for charging 12v batteries can be as small 10-watts and as large as 200-watts, but panels for 24v batteries begin at around 300-watts, minimum.
So, depending on your needs, you’ll need to get a 24v panel of at least 300-watts.
48v battery
When charging 48v batteries, you’re going to need a ton of power. These batteries hold roughly 5700-watt hours of power, and depending on your power usage you’ll need a lot of panel power to recharge the battery every day.
Ideally, you’ll need at least two kilowatts(2kWp) of panel power. This could come from eight 250-watt panels wired in series or five to six 350-watt panels.
50ah battery
A 100-watt panel is the best bet for a 50ah battery. You’ll be getting around 6 amps per hour (maximum), which will easily charge your battery in a day or less.
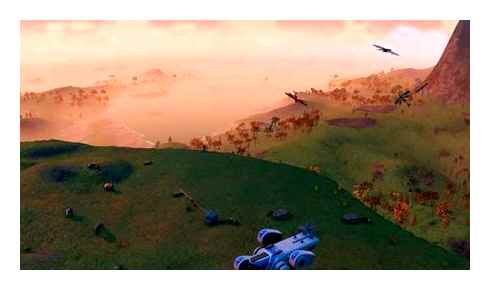
- 【Similar Usable Energy but 5 Times Faster Charging】LiTime 12V 50Ah LiFePO4 lithium battery has 640Wh energy.
- 【Top Protection 8 Times Lifespan】LiTime 12V 50Ah LiFePO4 battery is made of automotive grade LiFePO4 cells, which has.
- 【4 Times the Value for Money】LiTime 12V 50Ah LiFePO4 battery ensures you 5-year warranty while the best-selling lead-acid.
80ah battery
According to the formula mentioned above, you’d need a panel of between 120 and 140-watts to charge an 80ah battery in a day.
- TL1275/FP12750 Battery 12V 75AH Sealed Lead Acid Rechargeable Maintenance Free AGM Deep Cycle; Parcel: 1 x Battery (screws.
- Dimensions: 10.02 x 6.65 x 8.94 inches. High Performance, Long Service Life and Deep Discharge Recover.
- Fit For Wayne ESP25 Back-Up Pump, Electric Scooter, Golf Cart Electric DC, Solar Energy Storage, Pride Mobility Jazzy 1420.
100ah battery
While you could get away with a 100-watt panel as mentioned in our 1:1 ratio example, if you have a lot of power needs a 180-200 watt panel is best.
- MAINTENANCE FREE: 12.99x 6.73x 8.43 inches. WEIZE 12v 100Ah sealed lead acid battery is manufactured with absorbent glass.
- OPERATING TEMPERATURE: Charging Temp ranges from 14℉ (-10℃) to 122℉ (50℃), discharging Temp ranges from 5℉ (-15℃).
- LONGER LIFESPAN: Compared with flooded counterparts, its lower self-discharge of 1-3% per month allows long storage before.
120ah battery
For best results, a panel of around 210-watts is ideal for a 120ah battery but anything above 120 watts will do the trick too.
- 12 Volt 125Ah Group 31 AGM Deep Cycle Heavy Duty Battery
- Military grade custom made plates
- Float service life span of 8 to 10 years
140ah battery
Ideally, you’ll need around 250-watts to charge a 140ah battery in a day, but 150-watts and above is fine more most applications.
Batteries at the 140ah range are limited – it’s best practice to get the higher ah for high demand and longevity.
200ah battery
200ah is a lot of power, and your panel will be working hard to provide it. A panel of 350-watts is ideal, but 200-watts and above will manage too.
- High Quality Cells: LOSSIGY lithium iron phosphate batteries are manufactured of A-grade cells with higher energy density.
- 10-Year Lifespan: LOSSIGY Lifepo4 battery provides at least 4000 charge discharge cycles. The built-in BMS effectively.
- Easy Connectivity: LOSSIGY deep cycle lithium battery can be connected in series or in parallel with the same type batteries.
Solar panel battery sizes:
100-watt solar panel
Maximum 80-100ah, but ideally a 50ah battery.
200-watt solar panel
Ideally, a battery of 100-120ah but could work for a 150ah battery too.
300-watt solar panel
Best for 24v setups, and you’ll need a battery of at least 100ah to draw 1,000 watts or more, but a 200ah battery is ideal.
400-watt solar panel
Around 250ah of power, ideally a 200ah battery, or 2x120ah batteries.
500-watt solar panel
A 500-watt panel setup(2x 250-watt panels) can easily charge a 200ah battery in a day, so you could have 2x200ah batteries charging if you are not running them flat every day.
1000 watt solar panel
With 1,000 watts of panel power (4×250-watt panels, 3x 330-watt panels), you could easily get enough power to charge 2x200ah batteries, and probably three or even four if your energy usage is moderate.
- High Quality Cells: LOSSIGY lithium iron phosphate batteries are manufactured of A-grade cells with higher energy density.
- 10-Year Lifespan: LOSSIGY Lifepo4 battery provides at least 4000 charge discharge cycles. The built-in BMS effectively.
- Easy Connectivity: LOSSIGY deep cycle lithium battery can be connected in series or in parallel with the same type batteries.
Solar panel battery ratios:
When we start getting into large solar setups — 1kw and larger — you’ll need to start looking at 24v and 48v systems, which can handle the higher voltage loads.
For these large systems, 12v panels and inverters will not be sufficient. 12v, 24v panels, and 12v batteries can be used, but they’ll just need to be wired properly in series to bump up the voltage.
1kw solar system
This will depend on the individual panel sizes you opt for. As an example, you’ll need four 250-watt panels or three 330-watt panels.
The biggest single panel currently available is 615-watts, which you’ll need two of for a 1kw system.
Again, you’ll need to look at the load you need to run. If you’re running a 1kw continuous load, a 200ah battery will run for an hour, maximum.
Ideally, a battery bank of four 200ah batteries with 1kw of panels is best, or around 600ah of battery power.
2kw solar system
2kw of panels(8x 250-watt panels, 6x 330 panels, 3x 615-watt panels), and up to ten 200ah batteries.
4kw solar system
4kw of panels(12x 330-watt panels, 6x 615-watt panels), and 2,400ah of battery storage. Once you start getting into systems as large as 4kw, it’s best to go for lithium-ion batteries for power storage.
8kw solar system
8kw of panels (12x 615-watt panels), and 5,000ah of lithium-ion battery storage.
10kw solar system
10kw of panels (15x 615-watt panels), and 7,500ah of lithium-ion battery storage.
12kw solar system
12kw of panels (18x 615-watt panels), and 10,000ah of lithium-ion battery storage.
14kw solar system
14kw of panels (21x 615-watt panels), and 12,500ah of lithium-ion battery storage.
Choosing the right size
Choosing the right solar panel for your batteries depends on several factors, from your location to your energy needs, but there are some basic formulas you can follow.
Hopefully, we have helped remove some of the mystery and helped you work out the perfect panel and battery combination for your power needs!
How long do solar panels last?
How long solar panels last depends on their quality and how well looked after. In general, though, most solar panels will last between 25 and 30 years, with the most expensive models having a life expectancy of 40–50 years. That being said, solar panels will still produce energy after this time, although their capability will have declined significantly.
How do you set up a solar panel system?
The first step in setting up a solar system is determining how much power you need, and planning your solar system accordingly. After this, the setup is fairly straightforward;1. Gather all the required components together; panels, inverter, batteries, cables, etc. 2. Find a safe space in your home to house the inverter and batteries3. Fix the panels to your roof or a stand4. Connect the panels to your inverter or charge controller5. Connect your inverter to your batteries
What is required for solar panel installation?
Again, the first crucial step for any solar installation is calculating your power needs. Once you’ve done this, you’ll need to purchase the appropriate components. These include;1. Solar panels2. Batteries3. Inverter4. Charge controller5. Suitable cablesThese components are typically purchased separately according to your needs, but you can purchase ready-made solar kits that have all the components you need too.
Can I set up solar panels myself?
With a bit of basic DIY and electrical knowledge, you can certainly set up solar panels yourself, especially smaller systems. The setup can become somewhat complicated for large, high-power systems that are grid-tied, though, and you may want to get assistance from a qualified electrician.
Sol Voltaics is an affiliate and an Amazon Associate, we earn from qualifying purchases. at no extra cost to you.
Solar Power System (on-grid) project
Obtaining low-cost renewable solar energy has always had much appeal, but historically the investment costs has been rather off-putting, especially in the U.K. where it is perceived that the climate doesn’t provide a reliable enough amount of sunshine.
However, in recent years a number of things have changed this evaluation. Performance improvements in solar panels and associated power inverters have resulted gains in energy creation, coupled with the availability of modern battery arrays substantial enough to store the energy produced for later reuse. At the same time, shocks to world fuel have results in a Rapid shorting of the ‘payback period’; once it was considered that a typical household solar installation would take in the order of 25 years to recoup the investments costs. This has tumbled to around an estimated 8 years based on calculations made last year, and taking into account the recent price increases for domestic electricity supply, the period could be approaching 4 years with further shortening likely as energy continue to rise. The recent removal of VAT by the UK government on the implementation of solar energy systems is an added boost.
One additional further benefit that has recently arisen is the introduction by some power utility companies, such as Octopus Energy, of ‘agile’ export tariffs, which pay increased amounts at peak demand times. This can be taken advantage of by the use of Smart meters, supplying surplus generated or stored energy to the grid at the best times to maximise revenue, offsetting the purchase of electricity from the grid at other times.
Given that the future is anticipated to require increasing use of electricity to provide power for EV cars and hybrid vehicles, generating your own electricity makes increasing sense.
Overview of our implemented system
Given the now obvious benefits of a solar energy, we have acted accordingly and implemented a system, which has the following component parts:
- 13x 385W JA Solar Monocrystalline Panels with PERC technology, limited by the available roof space, but sufficient for energy needs.
- Alumero Mounting accessories Tigo Optimisers to enhance performance when part of the solar array is shaded.
- Luxpower Hybrid Auto Inverter, 16A single phase, to convert the generated 12V DC electricity to 240V AC for household consumption or export.
- 4.8 kW Aoboet Uhome battery storage array to store excess energy for later use.
- AC and DC isolators to connect the component system parts.
- Generation meter to measure energy production.
- Wi-Fi Monitoring portal for displaying instantaneous and historical performance.
The calculated annual yield for this system is 3,679kWh, which should be enough to fulfil the household’s electricity needs, estimated at 3,207kWh based on previous usage.
Solar Panels – the ‘heart’
Key to the collection of energy from the sun are naturally the solar panels. These vary in size, and technology is improving continuously, so the latest available are more efficient than previous generations.
Those selected for this project were 13 x 385W JA Solar Monocrystalline Panels with latest PERC (Passivated Emitter and Rear Cell) technology. Monocrystalline are more expensive but more efficient, with a longer lifespan than other types available. PERC technology improves light capture near the rear surface, optimising electrons flow and thereby achieving higher efficiencies.
The amount produced by a solar array naturally depends on sunlight hours and will be much lower with poor weather or as daylight reduces, whilst household electricity demand also varies during the day.
The ultimate aim of using solar power is to reduce as far as practically possible the need to source energy from the grid. Consequently, a larger array of modules than those just to meet the typical usage amount is needed to ensure adequate production whatever the weather, with the excess being stored or exported.
Alumero mounting kits were used for fixing the solar panels to the property roof, together with Tigo optimisers which maximise the generation from each panel. Without such optimisation, the power output from all solar modules can be reduced when some of the array is in shade.
DC isolators connect two ‘strings’ of series connected panels to the Hybrid Inverter.
Hybrid Inverter – ‘the brains’
In order for the system to be truly useful, power conversion and energy management functions are needed, to ensure a seamless and uninterrupted supply of electricity from the available sources i.e. an appropriate mix of the local generation, storage and grid supply. Chosen for this installation was a Lux Hybrid Automated 16 Amp single phase inverter.
The Hybrid Inverter ensures that when solar energy is available i.e. during daylight hours, this is firstly routed to provide for domestic consumption, and then used to charge the battery storage (as required, if not full). Any additional energy is exported to the external grid. When there is not enough energy generation from the solar array, the hybrid inverter routes the energy storage to the household, and when this is depleted, electricity is imported from the grid in the usual way. Critically, where to source electricity from is completely seamless such that the domestic consumption is never interrupted and the household is unaware of these ‘decisions’ being made.
It’s the inverter’s job to take the DC electricity produced by the solar panels and turn it into 240V AC electricity for household use. It’s a sad fact that many domestic appliances then take this 240V AC and convert it back to DC and lower voltages like 12V and 5V; this double conversion adding theoretical inefficiencies. But this is simpler to implement than rewiring the entire building and trying to then integrate with power to and from the grid.
AC Isolators connect the Inverter’s output to the household electricity supply.
Batteries – ‘the memory’
Quite literally, ‘saving for a rainy day’ is the function of the batteries, which add to the capability and capacity of solar power generation. They are effectively ‘optional’ since the system can be run without them. But since there is a huge natural variation between maximum sunlight and night-time, it makes sense to capture excess energy at peak times, and use this when sunlight is not available or sufficient.
Chosen for this project were 2x Aoboet Uhome-LFP 2400 providing 4.8kW of storage capacity.
At the beginning of a day, the batteries are naturally somewhat depleted, and therefore excess solar energy is initially used to charge them. Once full, they remain ‘on standby’ until later when generation is unable to fulfil the immediate electricity needs, in which case they start discharging their stored energy. Ideally, they will not become completely depleted over the course of the day and night, so that energy is not needed to be imported from the grid.
Grid – import / export
Electricity from the grid is the “insurance” for times when the solar energy is not able to fulfil demand. Naturally, this is likely to be due to a lack of winter daylight hours and/or poor weather, which of course has to be paid for.
But at other times, there will be an excess of energy that can be exported to earn back some of these costs. A Generation Meter as part of the solar energy system enables this export of electricity.
The bi-directional energy flow is measured with a ‘Smart’ meter using a suitable import / export tariff from the Utility company, such as the Octopus with their Agile tariff, and displayed on an associated Wi-Fi monitor.
As to be expected, the amount paid by the Utility for kWh export is considerably less than that charged for import, so it’s worth making best use of the generated and stored energy as much as possible, like running appliances when the sun shines!
An EPS (Emergency Power Supply) socket was additionally included in this project. Though optional, it was chosen for providing ‘backup power’ from the solar energy system in the event of a power outage from the grid supply. It is standard practice in such an event to shut off the export to the grid from solar energy systems to avoid difficulties whilst restoration work is in progress. But during such a period, the household can make use of the generated and stored local energy, for a limited time and restricted to a maximum of 13A. Avoiding excessive consumption, it should be possible to maintain a local supply for 12 hours, assuming a fully charged battery array.
To complete the project to become an ‘energy generator’ (as well as satisfying own consumption needs), an MCS (Microgeneration Certificate Scheme) certificate is issued, together with receiving acceptance documentation from the DNO (District Network Operative). This then allows the establishment of the export tariff with the Utility provider so that payments for excess energy exported will be made.
Operating performance
A Wi-Fi Portal provides the householder with an overview of the current operation of the solar energy system, displaying instantaneous status and historical energy performance for tracking generation yield and import / energy export.
Initially, it can be reported that average energy yield is around 0.86kWh, ranging between a typical peak of 2-4kW during the day and zero at night, compared with average consumption of approximately 0.35kWh, with the excess charging the batteries in the morning and exporting to the grid during the rest of the day. During the night, the consumption is met from the battery storage, with the batteries depleted to around 11% by the next day. It is noted that even during relatively cloudy days, at least around 10% of the 5kWh maximum power is generated, enough to at least meet the immediate consumption needs and even provide some battery replenishment.
A complete picture of the operating performance of the solar energy system will be known after a full year, taking into account the peak of summer and the shortest winter daylight period. Rising costs of electricity will also impact on the longer-term cost savings anticipated.
Conclusions
Hopefully this ‘project description’ is of interest and perhaps of use to anyone contemplating installing a Solar Energy system at their home or office premises. Please feel free to get in touch if you would like us to provide consultancy advice (on a no-obligation FOC basis) leading to a quotation for establishing your own system, or just to gain an in-depth appraisal and more information from our first-hand experience of implementing a Solar Energy system.
Our summary of conclusions at this stage having now implemented a system are:
- Solar energy collection has developed rapidly in recent years, particularly now that home energy storage is practical enough to capture excess energy during peak daylight and release it for use during the night or whenever demand exceeds generation.
- Although such systems are still a significant investment, given the recent escalation in energy costs, the ‘break-even’ point has reduced dramactically and the trend is for energy costs to continue to rise thereby making the payback period increasingly shorter.
- An attractive feature is the notion of being paid to supply energy to the grid, though it should be noted that currently at best this is 7.5p per kWh, so unlikely to be a significant revenue source. But it does mean that energy bills over the longer term will be vanishingly small.
- The contribution to the nation’s renewable energy mix helps in a small way to aid the drive to reduced carbon emissions and tackle climate change.
- Naturally, a suitable oriented roof or land space for solar panel installation is required, as well as a location for housing the inverter and batteries (loft space is ideal). Plus, it should be noted that a PV cable needs to be installed (most likely running down the outside wall of the building) to link the inverter to the consumer unit.
- Should power cuts from the grid occur in the future, the solar energy system is capable (thanks to the EPS socket) of providing power for a limited period to maintain household electricity use.
- With the increasing use of electric cars (all new will need to be at least hybrid by 2030), being able to source local renewable energy will make increasing sense.
@YellowsBestLtd our mission is in “Keeping Customers Operational”. We’re always keen to enhance our range of #business services, increase the #enterprise infrastructure we support and expand our mix of #sustainable solutions we offer for supply and maintenance of new and legacy #technologies and products for our customers.
Please help us understand what would be of interest to you by getting in touch to discuss your management services or solutions requirements, whether you’re implementing new systems or maintaining existing infrastructure networks to serve your operational business needs. We look forward to hearing from you.
thoughts on “Solar Power System (on-grid) project”
Nice write-up, Bernie. You’re right about the inefficiency of inverting the DC only to rectify it back to DC again. Specifically, this would be a case for EVs The charging of EVs is going to become increasingly prevalent; what can be done to provide a circuit that will allow an EV to charge at a higher rate than simply domestic 240v AC ?
Thanks for the feedback, Jon. Always happy to receive Комментарии и мнения владельцев. Take a look at my latest blog post, in which I’ve tried to expand on the points you have raised. https://www.yellowsbest.com/solar-energy-generation-applications-ac-vs-dc/ I hope this helps a bit in navigating the electric future!
Leave a Reply Cancel reply
Copyright © 2022 Yellows Best Limited. All Rights Reserved.